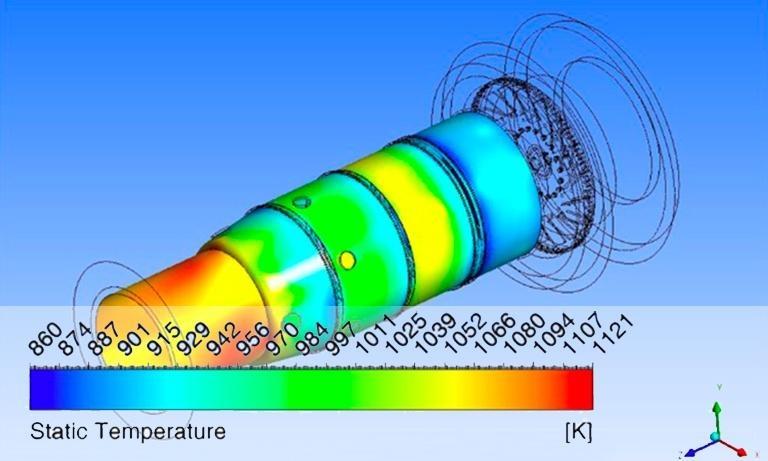
Hydrogen and the fuel-flexibility dilemma in gas turbines
DOI 10.12910/EAI2021-024
by Eugenio Giacomazzi - Head of Laboratory of Processes and Systems Engineering for Energy Decarbonization; Giuseppe Messina - Laboratory of Processes and Systems Engineering for Energy Decarbonization
The objective of this article is to clarify the state-of-the-art of gas turbines in terms of their fuel-flexibility, today intended as their capability to operate with hydrogen blends safely and reliably even with unplanned temporal composition fluctuations. From OEMs’s catalogues, DLE (Dry Low Emission) gas turbines can operate with natural gas/hydrogen mixtures up to 30% by volume of H2, on average: although this level corresponds just to 5% by mass with negligible reduction of CO2 (near 75% by volume of H2 is required to achieve a 50% CO2 reduction), it has to be considered as a challenging level in case of quick and unplanned hydrogen content fluctuations. Progress in fuel-flexibility is occurring fast but the journey towards fully-flexible “H2-turbines” (0-100% of H2) will still take some years and require some low TRL (2-3) activities too. The article reports the main problems to solve to reach the goal by 2030 in Europe and briefly describes ENEA’s activities on the topic. For example, ENEA’s IPSE Lab is developing and promoting activities on hydrogen gas turbines in some projects (Electric System Research, NextMGT), industrial collaborations (SNAM, BAKER HUGHES, ANSALDO ENERGIA) and international associations (European Turbine Network, Hydrogen Europe): focus is on the effects of adding H2 to natural gas in terms of flame topology (wrinkles, length), burning rate, thermo-acoustic instabilities, gas turbine operation
L’obiettivo di questo articolo è di chiarire lo stato dell’arte delle turbine a gas in termini della loro “fuel-flexibility”, oggi intesa come capacità di operare con miscele idrogenate in modo sicuro ed affidabile anche in presenza di inaspettate fluttuazioni temporali di composizione. Dalle informazioni deducibili dai cataloghi dei produttori, le attuali turbine a gas di tipo DLE (Dry Low Emission) sono in grado di operare con miscele gas naturale/idrogeno con un tenore di H2 fino al 30% in volume, in media: nonostante questo corrisponda al solo 5% in massa, con abbattimenti di CO2 irrilevanti (circa il 75% in vol. di idrogeno è richiesto per ottenere una riduzione di CO2 del 50%), tale livello è già considerevole in caso di rapide ed inaspettate variazioni temporali del contenuto di H2. I progressi nel campo della fuel-flexibility sono rapidi ma occorrono ancora alcuni anni ed attività di basso TRL (2-3) per ottenere turbine a gas in grado di operare con miscele con contenuto di idrogeno dallo 0 al 100%. L’articolo riporta i principali problemi da risolvere per raggiungere tale obiettivo in Europa entro il 2030 e sintetizza le attività ENEA sull’argomento. Per esempio, il Laboratorio IPSE di ENEA sta sviluppando e promuovendo attività sulle turbine a idrogeno in progetti (Ricerca di Sistema Elettrico, NextMGT), collaborazioni industriali (SNAM, BAKER HUGHES, ANSALDO ENERGIA) ed associazioni internazionali (European Turbine Network, Hydrogen Europe): l’attenzione è focalizzata sugli effetti dell’aggiunta di H2 al gas naturale in termini di topologia del fronte di fiamma (corrugamenti, lunghezza), velocità di combustione, instabilità termo-acustiche, operabilità delle turbine a gas.
Nowadays, the term fuel-flexibility refers to gas turbine capability to operate with hydrogen blends as fuel in a stable, safe and reliable way when the H2 content unpredictably varies in time due to intermittent production from renewables. Such blends range from hydrogen-enriched natural gas (HENG) to ammonia (a promising H2-carrier).
Both producers and users already have a huge experience in feeding their gas turbines with syngas containing hydrogen from 30 to 60% by volume, a solid base to burn mixtures with higher H2 concentrations. Looking at OEMs’ catalogues, the stat-of-the-art exhibits, on average, DLE (Dry Low Emission) gas turbines able to burn H2 up to 30% by volume with low NOx emissions (<25 ppmv at 15% O2). Due to the higher flame temperatures with respect to naural gas, in some cases NOx emissions are controlled only by derating the machine, i.e., reducing its power [1]; besides, it must be said that the strongest NOx limitations for natural gas (<15 ppmv at 15% O2) are not always reached today1. Higher hydrogen content can be burnt by adopting the WLE (Wet Low Emission) technology, but with penalties in NOx emissions, reduced efficiency and life cycle, higher costs. Hence, most of investments are on DLE technology, i.e., lean premixed combustion without dilution (steam, nitrogen, water), also for retrofit solutions. New combustion concepts are also explored, such as the sequential combustion at constant pressure (implemented in the GT36) and that based on micro-mixing and exhaust gases recirculation (EGR).
An important aspect, easy to understand but very often neglected is that large hydrogen mass fractions are required in fuel mixtures to significantly affect CO2 emissions. Talking in terms of volume fractions is a common practice, but in a methane/hydrogen mixture the H2 volume percentage largely differs from its mass counterpart, as shown in Fig. 1; for example, 30 and 50% vol. correspond just to 5 and 11% mass, while 80% vol. is required to reduce CO2 emissions by 55%. However, even the low 30% vol. becomes really challenging if significative temporal fluctuations of composition are considered.
Main issues
Hydrogen strongly alters combustion characteristics of a reacting mixture with respect to natural gas: the laminar flame speed increases, the ignition delay time decreases, the flammability limits enlarge, the adiabatic flame temperature increases, the turbulent flame speed becomes pressure dependent [2,3]. Such changes can dramatically affect combustion dynamics producing dangerous flashbacks [4] and/or thermos-acoustic instabilities [5,6] that may drive to unplanned overhauls thus reducing system reliability. Although both research and industrial sectors have been investigating the combustion instability topic for many years, it is still a not fully solved problem: strategies commonly adopted to avoid or limit the dangerous effects of such instabilities in modern gas turbines are based on empirical methods. Besides, the effects of hydrogen content on combustion dynamics make the Wobbe index unreliable for its applicability as fuel interchangeability index [7]. Special attention has to be given to control temperature peaks to limit NOx emission; maintaining them below 25 ppmv appears challenging for gas turbines operating in the range 0-100% H2 up to 2030: this is a difficult task due to the easily reached higher temperatures; there is an open discussion since the current limits for natural gas are lower, i.e., 15 ppmv. Another important effect of burning fuel mixtures with high H2 content is the higher steam content in the exhausts. This increases the heat exchange in the hot path of the gas turbine, thus requiring more cooling [8] and reducing component life cycle due to increased risk of hot corrosion. As a consequence, reducing the turbine inlet temperature, and consequently reducing the machine power with penalty losses of 2% points in efficiency (another challenging limit to maintain up to 2030), is a commonly adopted solution (see Fig. 2).
Adding H2 to natural gas also affects other operations of gas turbines, as at start-up and shut-down. Start-up procedures are commonly based on natural gas or liquid fuels, combining diffusive and premixed combustion at least up to FSNL2 state. At present, an average of 5% H2 by volume is tolerated at start-up.
Another term often used is “load-flexibility” or “operational flexibility”. This refers to gas turbine capability to change its power in small time to quickly stabilize the electric grid. Different solutions were proposed and implemented in the recent years, reaching today a power rate of 10% of nominal power per minute. Such a value seems to be fine for gas turbine users today and should be maintained in the future moving to hydrogen gas turbines.
With reference to micro-gas turbines, despite the efforts of some OEMs, they do not follow the learning curve of the heavy-duty ones at the same pace, having a very tiny hydrogen tolerance. However, being the micro-gas turbines users of the same gas grid of larger ones, more efforts on R&D are mandatory to reach the same hydrogen compliance. Looking at the gas turbine hydrogen tolerance on a broader perspective, nowadays and presumably at least up to 2030, the major issues will not be on the technology but on the hydrogen availability and cost. The current global production of hydrogen is around 70 Mt per year, 99% of them coming from coal and natural gas. If all current hydrogen production would be produced from water electrolysis, the amount of electrical energy required would be around 3600 TWh per year, more than the annual production of the whole European Union [10]. The availability issues will be much more challenging if the electricity for water electrolysis should come from the excess of VRES production: the amount of curtailed wind power generation in Germany on 2016 was 4722 GWh, while the energy requirement to produce hydrogen by electrolysis to run just one 9HA.02 gas turbine for 8000 hours at nominal power is 19600 GWh [11]. Even not introducing the costs issue, just from this perspective, in the authors’ opinion the use of blue hydrogen will be mandatory to build a realistic hydrogen value chain, as a precursor of a wide exploitation of green hydrogen when available in practical quantities for an effective CO2 emission abatement.
Research and development activities in ENEA
ENEA (IPSE Lab) is developing and also promoting activities on hydrogen gas turbines in some projects (Electric System Research, NextMGT), industrial collaborations (SNAM, BAKER HUGHES, ANSALDO ENERGIA) and international associations (European Turbine Network, Hydrogen Europe): focus is on the effects of adding H2 to natural gas in terms of flame topology (wrinkles, length), burning rate, thermo-acoustic instabilities, gas turbine operation.
Numerical simulations with the in-house code HeaRT run on the HPC platform CRESCO6 are aimed at flame topology analysis [12]: simple configuration injections are investigated also in EGR conditions, i.e., including mixing of fresh reactants with some hot exhaust gases. Fundamental studies are also performed experimentally in simple combustion devices suitably designed for a better coupling with numerical investigations [13]; in particular, the attention is focused on thermo-acoustics by using laser diagnostics and specific real-time sensors developed by ENEA (ODC, to identify instability precursors and DOES, to measure composition).
More complex geometries, like the combustor (including injection plate, liner, dilution holes) of the micro-gas turbine of the AGATUR plant in ENEA, are simulated by using the ANSYS code to obtain helpful information for the operation of the related gas turbine. An example of the effects of hydrogen in this combustor is in [14]. Work is in progress to feed the micro-gas turbine TURBEC T100 of the AGATUR plant with hydrogen [15,16]: here, the aim is to understand how to operate the machine and explore its behaviour under different steady and fluctuating H2 contents.
Perspectives
Hydrogen is going to play a central role in decarbonizing different sectors. In the power sector, gas turbines are asked to operate as a back-up service (seasonal and peak) to sustain variable renewable energy sources and stabilize the electric grid (both in voltage and frequency): their contribution to the electric system flexibility is expected to be important even in a 2040 projection (see Fig. 4), with an estimate of annual operating hours less than 3000. In this scenario, post-combustion carbon capture technologies are not applicable both from the technical and economic point of view: the potential annual CO2 reduction due to 100% hydrogen gas turbines is larger than 450 Mt.
To complete the picture and specialize it to Italy, it is reminded that natural gas is the major source contributing to limit the greenhouse gases emission, with very quick positive effects at local level. Besides, in Italy the gas network is very spread and reliable, while gas turbines are critical to ensure the adequacy of the electric system in view of the coal phase-out in 2025.
The recent European roadmap on gas turbines, developed within the Clean Hydrogen Energy association to be transferred into the “Strategic Research and Innovation Agenda” (SRIA 2020), has identified some goals that require also low TRL activities:
- development of fuel-flexible retrofit solution for existing power plants;
- research on combustion physics and dynamics for pure hydrogen and hydrogen blends (ammonia included) at gas turbine relevant conditions (TRL 2-3);
- development and demonstration of highly flexible combustion systems (0-100% H2);
- development and demonstration of innovative and advanced solutions up to their commercialization.
Conclusions
Although the long history of gas turbines and their application in different sectors, the article stressed that some research and developments are required for their reliable and safe operation with increasing hydrogen content, to reach at least 80% by volume for a significant CO2 reduction. However, behind the technological effort required, the widespread diffusion and availability of H2 is the real barrier, mainly linked to its production cost. Once accepted hydrogen as part of the solution to the climate change, transition to the “hydrogen society” can only happen starting to build the whole infrastructure and, in the authors’ opinion, by using the cheap “blue hydrogen” in the short term, and the still expensive “green hydrogen” in the long term.
REFERENCES
- ETN Global, Hydrogen Gas Turbines – The Path Towards a Zero-Carbon Gas Turbine, 2020.
- Kido H., Nakahara M., Hashimoto J., and Barat D., Turbulent Burning Velocities of Two-Component Fuel Mixtures of Methane, Propane and Hydrogen, JSME International Journal Series B 45(2): 355–362, 2002.
- Moccia V., D’Alessio J., Characterization of CH4-H2-Air mixtures in the high-pressure DHARMA reactor. Paper 287-503, 25th International Conference on Efficiency, Cost, Optimization (ECOS), 2012.
- Tuncer O., Acharya S., Uhm J.H., Dynamics and flashback characteristics of confined premixed hydrogen-enriched methane flames, Int. J. of Hydrogen Energy, 34: 496-506, 2009.
- Lieuwen T.C. and Yang V. editors, Combustion Instabilities in Gas Turbine Engines: Operational Experience, Fundamental Mechanisms, and Modelling, AIAA-book, Progress in Astronautics and Aeronautics, Volume 210, 2005.
- Abbott D., Practical examples of the impact of variations in gas composition on gas turbine operation and performance, Gas to Power Europe Forum, January 2012.
- Ferguson D., G.A. Richard, D. Straub, Fuel Interchangeability for Lean Premixed Combustion in Gas Turbine Engines, Proceedings of Turbo Expo: Power for Land, Sea and Air, ASME Paper No: GT2008-51261, pp. 973-981, 2009.
- P. Chiesa et al., Using Hydrogen as Gas Turbine Fuel, Journal of Engineering for Gas Turbines and Power, January 2005, Vol. 127 pp. 73-80.
- ANSALDO ENERGIA, Ansaldo Energia solutions for hydrogen combustion: fast-forward to a hydrogen fueled future,https://www.ansaldoenergia.com/PublishingImages/Idrogeno/Ansaldo%20Energia%20Solutions%20For%20Hydrogen%20Combustion.pdf, 2021.
- IEA, The Future of Hydrogen, Seizing today’s opportunities, Report prepared by the IEA for the F20, Japan, June 2019.
- Goldmeer J., GE Power, Power to Gas: Hydrogen for Power Generation – Fuel Flexible Gas Turbines as Enablers for a Low or Reduced Carbon Energy Ecosystem, GEA33861, February 2019.
- Cecere D., Giacomazzi E., Arcidiacono N.M., Picchia F.R., Direct Numerical Simulation of High Pressure Turbulent Lean Premixed CH4/H2–Air Slot Flames, Int. J. Hydrogen Energy, 43:5184-5198, 2018.
- Troiani G., Lapenna P.E., Lamioni R., Creta F., Experimental assessment of intrinsic instabilities in jet flames fed with hydrogen enriched hydrocarbon-air mixtures, European Combustion Meeting, April 2021.
- Bo A., Giacomazzi E., Messina G., Di Nardo A., Analysis of a fuel flexible micro gas turbine combustor through numerical simulations, J. of Engineering for Gas Turbines and Power, Transactions of the ASME, 140:1-10, 2018.
- Messina G., Pagliari L., Nobili M., Grasso A., Guidarelli G., Bo A., Attanasi S., Cassani S., Assettati A., Sviluppo e sperimentazione dell’assetto multi-fuel in una micro-turbina a gas TURBEC T100, Report Ricerca di Sistema Elettrico RdS/PAR2015/184, ENEA, September 2017.
- Messina G., Specifiche per la realizzazione della rampa H2 dell’impianto AGATUR, Report Ricerca di Sistema Elettrico RdS/PTR2019/133, ENEA, December 2019.
- IEA, World Energy Outlook, 2019.